Medical Physicists Bring New Value to Patient-Centered Care
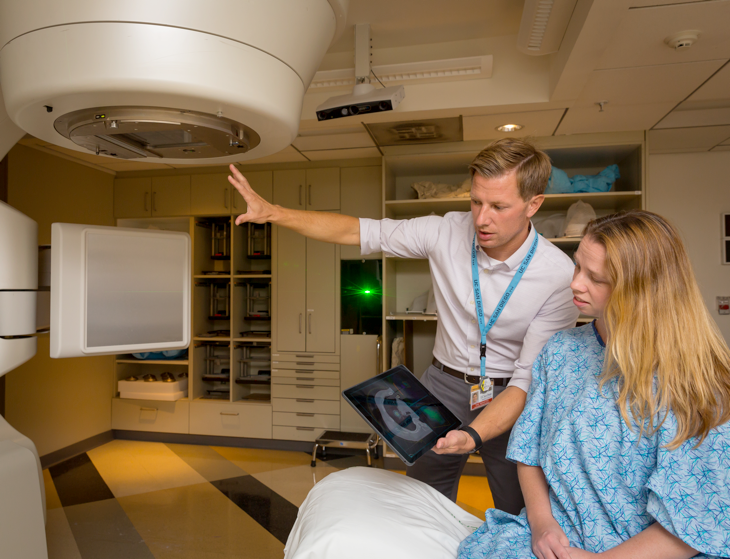
By Ehsan Samei, PhD
Until recently, medical physicists were mainly occupied with inspecting the quality and safety of imaging and therapeutic equipment in radiology and radiation oncology to ensure they met specifications and to protect staff and patients from exposure to X-ray radiation, electromagnetic fields, and gamma rays. They managed equipment such as linear accelerators, CT scanners and MRI equipment.
Today, at the University of California San Diego Health’s Moores Cancer Center and elsewhere, radiation oncology medical physicists do more than manage hardware and develop protocols. “We meet with patients at their consult or before their CT simulation to establish a relationship and provide an overview of their care,” says Todd Atwood, PhD, a medical physicist and assistant professor at UC San Diego School of Medicine. “Then we meet with patients again prior to their first treatment to review their treatment plan and discuss their radiation delivery. We encourage them to contact us directly during the course of their treatment with any technical questions that may arise.”
This is a significant departure from the norm. At many hospitals, the medical physicist is unknown to patients. Physicians and staff in radiology and radiation oncology may know one, but only as their physics teacher. Physicians from other specialties, nurses and other healthcare staff are much less likely to be familiar with—or even aware of—the existence of medical physicists, let alone the value that they provide.
That is changing quickly.
Faced with broad and profound changes in the delivery of healthcare—including declining reimbursements and new mandates to deliver value-based, personalized, and evidence-based medicine—healthcare is benefiting from the growing contributions of medical physicists. These practitioners have strong analytical and problem-solving skills; technical expertise in physics and biomedicine; and knowledge of clinical processes and workflow, including imaging science and radiation therapy planning and delivery.
Today’s medical physicists reflect on how the work they do contributes to patient care, and communicate that value by engaging routinely with diverse members of the healthcare team. Regularly participating in such interdisciplinary functions as tumor boards, chart rounds, and quality and safety committees allows medical physicists to create awareness of their capabilities and appreciation for their efforts.
Optimizing imaging dose and quality through monitoring
Perhaps the most well-known value proposition for medical physics today is in achieving a balance between radiation dose and image quality in CT, an effort that involves more personalized care and greater interaction with patients.
The use of CT scans nearly tripled from 1996 to 2010, according to a study based on data from patients in six large HMOs (Smith-Bindman et al., 2012). The researchers found that 2.5% of the patients were exposed to 20–50 millisieverts of radiation in 2010, a level that the International Commission on Radiological Protection says is excessive. Another 1.4% of the patients were exposed to more than 50 millisieverts of radiation, which the U.S. Nuclear Regulatory Commission says is unsafe. About 10% of CT examinations are performed in children, who have generally higher sensitivity to radiation due to growing tissues (Dorfman et al., 2011; Frush & Applegate, 2004; Wildman-Tobriner et al., 2014). A study from the National Institutes of Health showed a correlation between CT scans in children and their subsequent risk of developing cancer (Pearce et al., 2012).
These potential risks have garnered a great deal of attention in recent years from scientists, regulators, and the public. There has been a call for accountability regarding both the frequency of medical imaging examinations involving ionizing radiation and the magnitude of radiation exposure involved.
In 2009, the National Institutes of Health began recording doses for CT in patients’ medical records. Since July 2015, The Joint Commission has mandated that providers document radiation dose on every study produced during a CT examination. The radiation dose must be exam-specific, summarized by series or anatomic area, and documented in a retrievable format. The provider must also review incidents where the radiation dose emitted by the CT imaging system during diagnostic exams exceeded expected dose ranges identified in imaging protocols.
Some state regulations have also been instituted. Since July 2012, California has included CT dose estimation in the patient report. Texas also enacted a law requiring dose monitoring.
The amount of radiation from a single CT exam is considered low-level exposure, generally below about 50–100 millisieverts. A high dose of radiation, well beyond the low-level range, is an established carcinogen in humans and can have other biological consequences such as cataracts, epilation, and skin erythema. The threshold for and potential magnitude of risks for cancer from low-level radiation are strongly debated within the scientific and medical communities, but there are four points of consensus:
- For an individual patient, the risk associated with a typical imaging exam is very small
- Even with a small risk, the magnitude of CT utilization raises concerns about population risk
- The risk, regardless of how uncertain it might be, is higher for children
- The risk, regardless of how uncertain it might be, should be minimized
A measurement conundrum
Complicating this picture is that there is no one agreed-upon metric of radiation, and each one used provides a different (and differently limited) representation of factors contributing to radiation burden. In CT, the most commonly implemented metric is volume CT dose index, or CTDIvol. This reflects the radiation output of a CT system in units of dose to a standard-sized object, akin to a test dummy. While effective in characterizing the system output for CT protocols in terms of dose, CTDIvol fails to represent all protocols or to fully account for individual patient attributes (McCollough et al., 2011). Differences in body size between large and small patients alone can result in up to a 10-fold difference in actual radiation dose.
The size-specific dose estimate (SSDE) was developed to address one of CTDIvol’s major limitations by factoring a patient’s overall size into the estimated dose. While better than CTDIvol, SSDE still does not account for body shape, organ locations and orientation, tissue composition, patient’s age and gender, or other technical factors. And after all, these metrics are technology-focused, while quantities of patient radiation exposure should be reflective of the burden to the patient. This is the only way that radiation risk can be put in context of other risks and benefits associated with medical intervention.
Further complicating matters, larger medical institutions often use multiple CT systems of different makes and models. The imaging protocols across the systems are not necessarily consistent, nor are they applied consistently.
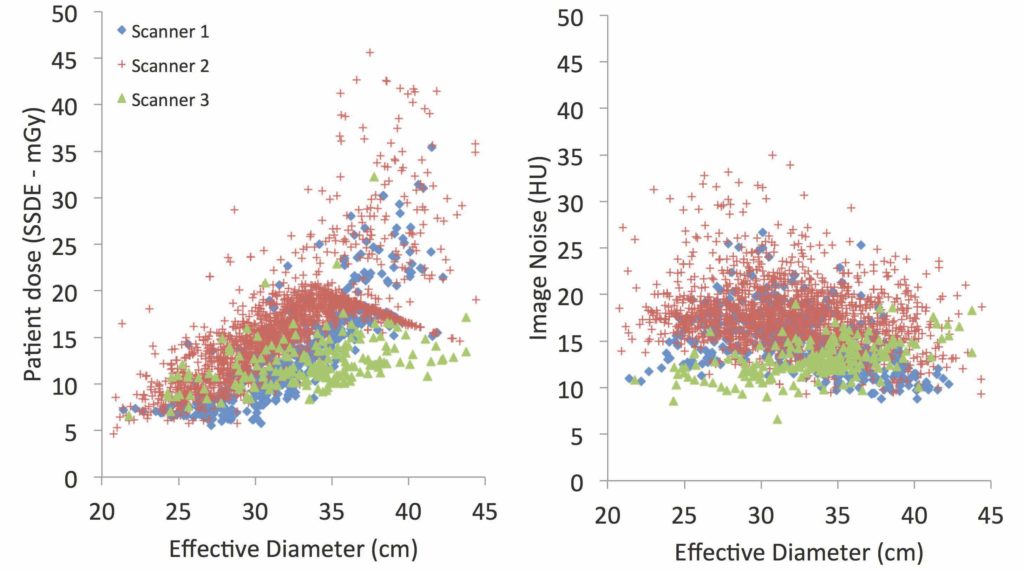
Proper medical imaging requires a careful balance between the quality and the safety of the exam. Too low a dose and the image will be of poor resolution; too high a dose and safety can be undermined. Five years ago, the imaging physics team at Duke University in Durham, North Carolina, began looking at radiation dose values in patients undergoing imaging examinations and found that to optimize the balance between dose and image quality, the entire imaging chain needed to be evaluated.
Historically, radiation dose monitoring has been about aggregating dose data without regard to patient size, age, gender, body type, or resultant image quality. The only question that is normally asked of the dose monitoring system is whether the dose for a given exam exceeds some global threshold. “We are different in that we are asking more and targeted questions making sure they take into account patient-specific factors such as size,” says Joshua Wilson, a physicist at Duke. “A large patient may require a high dose to achieve necessary image quality, which appears as an ‘overdose’ and requires unnecessary follow-up, while a small patient could receive two or three times what’s necessary for a good image and yet be well under the threshold.” Also, the purpose of the examination would also need to be taken into consideration. The image quality and thus dose needed for detecting a faint tumor in the liver is higher than what would be needed to detect a kidney stone.
The Duke team’s approach is automating objective measures of image quality. “Beyond just compiling data on patient demographics, protocol parameters, and resultant radiation doses, we are compiling data on image quality that relates back to human observer preferences,” Wilson says. This way, when radiologists, technologists, and medical physicists meet to discuss imaging protocols, parameter modifications can be driven by objective, quantitative data rather than anecdotes.
A direct role in patient care
This level of clinical interaction and intentional engagement can also impact the therapeutic processes, leading to better-informed treatment decisions. Together with the surgeon, medical oncologist, and radiation oncologist, the medical physicist thus assumes an essential role on the treatment team—ensuring each patient receives the best possible treatment, the least radiation side effects, and a less stressful experience.
As part of the team, medical physicists continually strive to incorporate new physics discoveries into highly personalized and more effective treatment plans. Working with radiation oncologists, they ensure cancer patients receive the prescribed dose of radiation therapy, targeted to the cancerous cells while protecting healthy tissue. This role is crucial to ensure precise, individualized delivery of radiation, which should be the case with any form of treatment. In imaging, radiation is a side effect that need to me monitored and managed. In radiation therapy, radiation is intentionally used for treatment. Simplified, one might think of radiation therapy as a combination of localized chemotherapy and incisionless surgery. But this radiation cannot be delivered to the tumor without also irradiating the healthy tissue. This is where a medical physicist steps in, maximizing the wanted radiation and minimizing the unwanted ones. These outstanding benefits of radiation treatment cannot be realized without medical physicists.
For more than a decade, Massachusetts General Hospital in Boston has focused on providing the lowest dose of radiation for each patient. Among other things, Mass General developed computerized provider order entry and support systems to assist physicians in determining whether conducting a study is in a patient’s best interest.
When placing an order, physicians receive a utility score for the test, along with scores for potential alternate tests, to determine which test is best based on the patient’s age, size, gender, and medical condition. “If there is an alternative test, we will try that first,” says Dushyant Sahani, MD, director of CT for Mass General. The system also alerts physicians if similar tests have been ordered and performed to avoid duplication of imaging procedures. Safety checks play an important role. Prior to conducting imaging exams, patient information is checked and double-checked to guarantee that the exam is absolutely necessary. Physicists provide the comparative assessment that informs these choices.
Making MRI safe for patients with stimulators
Medical physicists at University Hospitals Cleveland Medical Center were intimately involved in a project seemingly far afield from their area of specialization: facilitating the evaluation of epilepsy patients for curative surgery.
Epilepsy is typically managed via medication, but many patients also receive implanted nerve stimulators to help control their symptoms. A procedure to cure certain types of epilepsy was developed by a surgeon in the Division of Pediatric Epilepsy at University Hospitals, but it came with a big drawback: The surgery requires MRI for pre-surgical evaluation and planning to determine whether a patient can benefit from it.
“Our hospital had a strict policy prohibiting MRI of patients with any kind of active implanted electrical or electronic devices—including nerve stimulators,” says David W. Jordan, senior diagnostic medical physicist for University Hospitals.
At the time, the only way to determine whether a patient with a stimulator could have the curative surgery would have been to surgically remove the stimulator before a MRI scan. “This was unacceptable to the epilepsy team, because it would involve removing many working stimulators … and those patients who turned out to not be surgical candidates would now be left without a working stimulator to manage their epilepsy symptoms,” Jordan says.
To find a solution, Jordan created a working group that brought together the epilepsy team, radiologists, and the manufacturer of the stimulator to evaluate potentially safe MRI approaches for these patients. He worked with the stimulator company to review all of their information and data about MRI safety conditions and tests for their devices. At the same time, he determined the technical capabilities of the hospital’s various MRI scanners, and presented the device and scanner information to the radiologists.
“We reviewed possible MRI approaches that would satisfy the documented safety conditions for implants,” says Jordan. “Then I worked with the epilepsy team to explain the features and limitations of our MRI scanners to ensure our approach would suit their needs. With agreement from both sides, we proposed an updated implant policy to the hospital’s risk managers.”
Now, the epilepsy team has a “feasible approach to evaluate stimulator-managed patients for curative surgery, and in the process, our MRI department became a referral center for stimulator patients who are unable to get MRI studies at other centers,” he adds. “And the stimulator manufacturer is using our experience as a case study to help other centers set up programs for safe MRI scanning for their stimulator patients.”
Conclusion
More than ever, medical physicists have a clear mandate to put their expertise to use in centering medical procedures around the patient, using the science of medical physics to provide precise, consistent, and optimal care.
“Our goal is to improve patient care while establishing a brand-new channel of clinical collaboration with our physician colleagues,” says Derek Brown, PhD, associate professor of radiation medicine and applied sciences at University of San Diego Health. “We see a future when the radiation oncology medical physicist will develop an independent, professional relationship with patients.” In both imaging and therapy, medical physicists bring unique value to healthcare, enabling innovative, personalized precision care through clinical application of physical sciences.
About the author
Dr. Ehsan Samei is a professor at Duke University and the chair of the Medical Physics 3.0 initiative of the American Association of Physicists in Medicine.
REFERENCES
Dorfman, A. L., Fazel, R., Einstein, A. J., Applegate, K. E., Krumholz, H. M., Wang, Y. … Nallamothu, B. K. (2011). Use of medical imaging procedures with ionizing radiation in children: A population-based study. Arch Pediatr Adolesc Med, 165(5), 458–464.
Frush, D.P., & Applegate, K. (2004). Computed tomography and radiation: Understanding the issues. J Am Coll Radiol, 1(2), 113–119.
McCollough, C. H., Leng, S., Yu, L., Cody, D. D., Boone, J. M., & McNitt-Gray, M. F. (2011). CT dose index and patient dose: They are not the same thing. Radiology, 259(2), 311–316.
Pearce, M. S., Salotti, J. A., Little, M. P., McHugh, K., Lee, C., Kim, K. P … de González, A. B. (2012). Radiation exposure from CT scans in childhood and subsequent risk of leukaemia and brain tumours: A retrospective cohort study. Lancet, 380(9840), 499–505.
Smith-Bindman, R., Miglioretti, D. L., Johnson, E., Lee, C., Feigelson, H. S., Flynn, M. … Williams, A. E. (2012). Use of diagnostic imaging studies and associated radiation exposure for patients enrolled in large integrated health care systems, 1996-2010. JAMA, 307(22), 2400–2409.
Wildman-Tobriner, B., Chatfield, M., Behnke, E. S., & Frush, D. P. (2014, December 4). ACR Dose Index Registry: A resource for pediatric CT practice assessment and radiation dose estimations. Paper presented at the Radiological Society of North America Annual Meeting, Chicago.